Post
Dying of the Light
31 March 2013
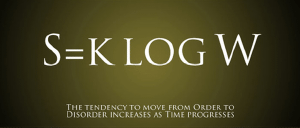
I enjoy a good cup of coffee in the morning. It’s particularly nice on cool morning, sipping the coffee while the heat of the cup warms my hands. Life is good.
Of course that’s always how it works. The coffee is initially warmer than my hands, so as I hold the cup the heat flows from the coffee into my hands. But why does it always work that way? It seems rather obvious. That’s just what heat does. If you put something hot next to something cold, the heat will flow from the hot object to the cold object until the two have reached the same temperature. This is why my coffee cools to room temperature over time. But this property of heat has some interesting consequences. Consequences that may determine the fate of the universe.
In the 1700s, heat was thought to be caused by a kind of fluid known as caloric. It is where the term calorie comes from. A hot object was thought to possess a lot of caloric. A basic property of caloric was that it tended to spread out as much as possible. So if you placed something cold (with not much caloric) against something hot (with lots of caloric), the caloric would flow from the hot object to the cold until it was evenly spread out. Thus the hot object loses caloric and cools, while the cold object gains caloric and warms.
This idea isn’t bad as basic theories go, but there were some things it couldn’t explain. One was things like rubbing your hands together. If you rub your hands together, they get warmer. Does that mean the motion of your hands somehow draws caloric to them? Another was exothermic reactions. You can set a cold log on fire, and it produces a lot of heat. So where was all the caloric if the log was cold?
Then in 1845 James Joule demonstrated that heat was a form of energy. It was known that heat could be used to do mechanical work (such as with a steam engine), but Joule showed that you could also convert mechanical work into heat. Heat and work were therefore two types of energy. Because of his research, the modern unit of energy is the Joule. This connection between heat and mechanical work soon led to the development of three basic laws of thermodynamics (heat behavior).
The first law is simply that energy in all its forms is conserved. This can be heat energy, mechanical work, energy of chemical reactions, etc. Energy can move from one form to another, but it can’t be created or destroyed. The second law states that things will always move toward thermodynamic equilibrium. Stated simply, it means heat will always flow from hot to cold. The third law is stated in a number of ways, but basically says there is a limit to how cool something can be, and that limit is known as absolute zero.
Of these rules, the second law of thermodynamics is perhaps the most interesting and misunderstood. We know that heat flows from hot to cold, but why? If I set a room-temperature cup of coffee on the table, why doesn’t the coffee spontaneously get warmer by cooling the cup? If the cup cooled and put all that heat into the coffee, you could have piping hot coffee in an ice cold up, and energy would still be conserved. Why does that never happen? Likewise, if you set a cup of hot coffee on the table, why does it cool down? If the coffee kept all its heat, energy would be conserved. Why does the heat always flow from the hot coffee to the surrounding cool air?
Regardless of the mechanism, the second law of thermodynamics has some specific consequences. One has to do with using heat to do work. Suppose you wanted to make a steam engine. There are lots of ways to do this, but they all boil down to a basic process. For example: heat a volume of steam, let it expand doing mechanical work, let the steam cool, then compress it back to its original volume and heat it again.
From the first law of thermodynamics you are not creating energy, you are simply transforming heat energy into work energy. But from the second law of thermodynamics, you can’t convert all of the heat to work. In the example above, you have to let the expanded steam cool before you compress it back to its original volume. If you don’t let it cool, then it would take just as much energy to compress the steam as you got by letting it expand. But when you let the steam cool, the heat it releases is just wasted energy. To get work from your steam engine, some of your energy is wasted.
This wasted energy is known as entropy. So the second law of thermodynamics says that you can convert heat into work, but you can’t convert all of the heat to work. Some of the original heat will become entropy. This is true for more than simple steam engines. For this reasons, the second law of thermodynamics is often stated as the fact that you can never do something with 100% efficiency, or more formally “the entropy of a system can never decrease.”
Because of this, entropy is often expressed as the unusable part of a system, and this leads to a lot of misconceptions. For example, there are those who state that the second law means that evolution can’t be true. If the “unusable” portion of a system always increases, then it is surely impossible for simple cells to become complex humans. That would be true if it were not for the Sun. The Sun creates lots of usable energy for life on Earth, as well as lots of entropy. Life can evolve on Earth because of the Sun. The total entropy of the Sun, Earth and its living organisms continues to increase over time, even as life evolves. The same argument could be made for a refrigerator. If heat flows from hot to cold, then how can a fridge get cooler? The answer is that the heat engine of the fridge uses energy to compress a fluid causing it to heat up. It lets the compressed fluid cool to room temperature, then it expands the fluid causing it to cool, which it uses to cool the interior of the fridge. A refrigerator can move heat from cold to hot, but it must use energy to do so, and it creates more waste heat than it removes from the fridge. Entropy still increases. The next time someone uses thermodynamics to deny evolution, explain to them that by the same argument their refrigerator shouldn’t exist.
Of course this definition for entropy is a bit nebulous. The problem is that when entropy was first defined there wasn’t a good understanding of how materials are made of atoms and molecules. Throughout most of the 1800s, the “atomist” view of matter was controversial. In 1808, John Dalton demonstrated that materials were made of varying ratios of chemical elements, and proposed an atomic theory of matter. While this theory was widely accepted by chemists, it was less accepted by physicists and those who studied thermodynamics.
Then in the late 1800s Ludwig Boltzmann developed a kinetic theory of gases. He proposed that the properties of a gas, such as its temperature and pressure, were due to the the motion and interactions of atoms and molecules. This had several advantages. For example, the hotter a gas, the faster the atoms and molecules would bounce around, therefore temperature was a measure of the kinetic (moving) energy of the atoms. The pressure of a gas is due to the atoms and molecules bouncing off the walls of the container. If the gas is heated, the atoms move faster and bounce off the container walls harder and more frequently. This explains why the pressure of an enclosed gas increases when you heat it.
Boltzmann’s kinetic theory not only explained how heat, work and energy are connected, it also gave a clear definition of entropy. The pressure, temperature and volume of a gas is known as the state of the gas. Since these are determined by the positions and speeds of all the atoms or molecules in the gas, Boltzmann called these the microstate of the gas (the state of all the microscopic particles). For a given state of the gas, there are lots of ways the atoms could be moving and bouncing around. As long as the average motion of all the atoms is about the same, then the pressure, temperature and volume of the gas will be the same. This means there are lots of equivalent microstates for a given state of the gas.
Boltzmann proposed a connection between the entropy of a system and the number of equivalent microstates, as seen in the equation above. In the equation, S is the entropy of the system, K is the number known as Boltzmann’s constant, W is number of equivalent microstates, and LOG represents the natural logarithm. What the equation says is that the entropy of a system in a particular state depends on the number of equivalent microstates that state has.
But how do equivalent microstates relate to heat flowing from hot to cold? Imagine an ice cube in a cup of warm water. The water molecules in the ice cube are frozen in a crystal structure. This structure is pretty rigid, so there aren’t a lot of ways for the water molecules to move. This means the number of equivalent microstates is rather small. As the ice melts the crystal structure breaks down, and the water molecules are much more free to move. This means there are many more equivalent microstates for water than for ice. So heat flows into the ice, which increases the number of equivalent microstates, so the entropy of the system increases. The second law of thermodynamics applies both ways.
This has a very clear consequence for the universe. In the earliest moments of the universe, immediately after the big bang, the number of possible states that could describe the universe was likely very small. This means the entropy of the universe was very low. Since the second law of thermodynamics says entropy can never decrease (but can increase), over time the entropy of the universe has increased, and its entropy will continue to increase. But a consequence of this is that every cosmic process does what it does at the cost of increasing the entropy of the universe. Gravity can coalesce clouds of hydrogen and helium into stars, but some heat energy will be wasted. Stars can fuse hydrogen into higher elements, but it does so by releasing light and heat into the cosmos. Some of that light and heat may warm planets. Life can use that light and heat to evolve, but the star will eventually use up its useful energy. Some stars will explode, and new stars form from the ashes, but none of this is perfectly efficient. The entropy of the universe will continue to increase. The stars will cool, the universe will expand. Eventually even the black holes will radiate away their mass into a vast, dark and cold universe. Entropy cannot decrease. The second law of thermodynamics means that there will come a time when the light of the last star fades. The dying of the light.
The second law also says that heat flows from hot to cold. The warm cup of coffee in my hands tells me not only that life is good, but that life is short. The physics that drives the heat of the coffee into my hands also drives me, you, the Sun, and the universe toward their inevitable end.
This end is known as the heat death of the universe. There is still debate as to whether it is an accurate description of the fate of the universe. There’s still a great deal we don’t understand about entropy, much less the universe as a whole. But it is a real possibility. The universe has a beginning, and it could well have an end.
Sometimes what we discover about the universe can be unsettling, even terrifying. The universe is massive, complex, and subtle. It is easy to look upon its majesty and despair.
Or we can stand together on our small planet, and look out into the night in wonder. We can recognize that we few, we happy few, have a true understanding of what the universe is.
The universe is a wondrous thing.
And it is ours to explore.
Missed the beginning of this series? The introduction starts here.